Stress granules are shock absorbers that prevent excessive innate immune responses to dsRNA
- PMID: 37028415
- PMCID: PMC10170497
- DOI: 10.1016/j.molcel.2023.03.010
Stress granules are shock absorbers that prevent excessive innate immune responses to dsRNA
Abstract
Proper defense against microbial infection depends on the controlled activation of the immune system. This is particularly important for the RIG-I-like receptors (RLRs), which recognize viral dsRNA and initiate antiviral innate immune responses with the potential of triggering systemic inflammation and immunopathology. Here, we show that stress granules (SGs), molecular condensates that form in response to various stresses including viral dsRNA, play key roles in the controlled activation of RLR signaling. Without the SG nucleators G3BP1/2 and UBAP2L, dsRNA triggers excessive inflammation and immune-mediated apoptosis. In addition to exogenous dsRNA, host-derived dsRNA generated in response to ADAR1 deficiency is also controlled by SG biology. Intriguingly, SGs can function beyond immune control by suppressing viral replication independently of the RLR pathway. These observations thus highlight the multi-functional nature of SGs as cellular "shock absorbers" that converge on protecting cell homeostasis by dampening both toxic immune response and viral replication.
Keywords: ADAR1; RIG-I-like receptor; antiviral signaling; dsRNA; immune-mediated apoptosis; immunopathology; innate immunity; integrated stress response; molecular condensate; stress granule.
Copyright © 2023 The Authors. Published by Elsevier Inc. All rights reserved.
Conflict of interest statement
Declaration of interests B.t. is a cofounder of Archean Biologics. S.H. is a consultant for Odyssey therapeutics and CJ Cheil Jedang.
Figures
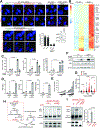
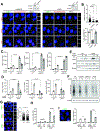
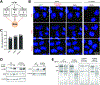

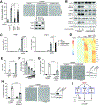
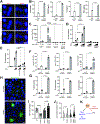
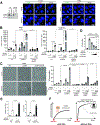
Similar articles
-
UBAP2L Forms Distinct Cores that Act in Nucleating Stress Granules Upstream of G3BP1.Curr Biol. 2020 Feb 24;30(4):698-707.e6. doi: 10.1016/j.cub.2019.12.020. Epub 2020 Jan 16. Curr Biol. 2020. PMID: 31956030
-
Enterovirus 71 inhibits cytoplasmic stress granule formation during the late stage of infection.Virus Res. 2018 Aug 15;255:55-67. doi: 10.1016/j.virusres.2018.07.006. Epub 2018 Jul 10. Virus Res. 2018. PMID: 30006004
-
The association of UBAP2L and G3BP1 mediated by small nucleolar RNA is essential for stress granule formation.Commun Biol. 2023 Apr 14;6(1):415. doi: 10.1038/s42003-023-04754-w. Commun Biol. 2023. PMID: 37059803 Free PMC article.
-
A closer look at mammalian antiviral condensates.Biochem Soc Trans. 2024 Jun 26;52(3):1393-1404. doi: 10.1042/BST20231296. Biochem Soc Trans. 2024. PMID: 38778761 Free PMC article. Review.
-
Rasputin a decade on and more promiscuous than ever? A review of G3BPs.Biochim Biophys Acta Mol Cell Res. 2019 Mar;1866(3):360-370. doi: 10.1016/j.bbamcr.2018.09.001. Epub 2018 Sep 5. Biochim Biophys Acta Mol Cell Res. 2019. PMID: 30595162 Free PMC article. Review.
Cited by
-
The human disease-associated gene ZNFX1 controls inflammation through inhibition of the NLRP3 inflammasome.EMBO J. 2024 Nov;43(22):5469-5493. doi: 10.1038/s44318-024-00236-9. Epub 2024 Sep 27. EMBO J. 2024. PMID: 39333773 Free PMC article.
-
RNA structures within Venezuelan equine encephalitis virus E1 alter macrophage replication fitness and contribute to viral emergence.PLoS Pathog. 2024 Sep 27;20(9):e1012179. doi: 10.1371/journal.ppat.1012179. eCollection 2024 Sep. PLoS Pathog. 2024. PMID: 39331659 Free PMC article.
-
Mitochondrial double-stranded RNA homeostasis depends on cell-cycle progression.Life Sci Alliance. 2024 Aug 29;7(11):e202402764. doi: 10.26508/lsa.202402764. Print 2024 Nov. Life Sci Alliance. 2024. PMID: 39209534 Free PMC article.
-
Metal-polyphenol-network coated R612F nanoparticles reduce drug resistance in hepatocellular carcinoma by inhibiting stress granules.Cell Death Discov. 2024 Aug 28;10(1):384. doi: 10.1038/s41420-024-02161-6. Cell Death Discov. 2024. PMID: 39198406 Free PMC article.
-
CRISPR perfect adaptation for robust control of cellular immune and apoptotic responses.Nucleic Acids Res. 2024 Sep 9;52(16):10005-10016. doi: 10.1093/nar/gkae665. Nucleic Acids Res. 2024. PMID: 39087566 Free PMC article.
References
Publication types
MeSH terms
Substances
Grants and funding
LinkOut - more resources
Full Text Sources
Miscellaneous