User:Marshallsumter/Radiation astronomy1/Fluorescences

Many samples of fluorite exhibit fluorescence under ultraviolet light, a property that takes its name from fluorite.[1] Many minerals, as well as other substances, fluoresce. Fluorescence involves the elevation of electron energy levels by quanta of ultraviolet light, followed by the progressive falling back of the electrons into their previous energy state, releasing quanta of visible light in the process. In fluorite, the visible light emitted is most commonly blue, but red, purple, yellow, green and white also occur. The fluorescence of fluorite may be due to mineral impurities such as yttrium, ytterbium, or organic matter in the crystal lattice. In particular, the blue fluorescence seen in fluorites from certain parts of Great Britain responsible for the naming of the phenomenon of fluorescence itself, has been attributed to the presence of inclusions of divalent europium in the crystal.[2]
Idrialites
[edit | edit source]
Def. a "soft, orthorhombic hydrocarbon [C22H14] mineral, usually greenish-yellow to light brown in colour with bluish fluorescence"[3] is called an idrialite.
Continua
[edit | edit source]X-ray continuum emission can arise both from a jet and from the hot corona of the accretion disc via a scattering process: in both cases it shows a power-law spectrum. In some radio-quiet active galactic nuclei (AGN) there is an excess of soft X-ray emission in addition to the power-law component.
X-ray line emission is a result of illumination of cold heavy elements by the X-ray continuum that causes fluorescence of X-ray emission lines.
Using X-rays to determine a crystal structure results in diffraction intensities that are represented in reciprocal space as peaks. These have a finite width due to a variety of defects away from a perfectly periodic lattice. There may be significant diffuse scattering, a continuum of scattered X-rays that fall between the Bragg peaks.
The X-ray continuum can arise from bremsstrahlung, black-body radiation, synchrotron radiation, or what is called inverse Compton scattering of lower-energy photons by relativistic electrons, knock-on collisions of fast protons with atomic electrons, and atomic recombination, with or without additional electron transitions.[4] During more than a decade of observations of X-ray emission from the Sun, evidence of the existence of an isotropic X-ray background flux was obtained in 1956.[5]
Ultra-high-energy cosmic rays
[edit | edit source]The objective of the mission is the observation of gamma-ray bursts, high-energy cosmic rays and transient phenomena in the Earth's upper atmosphere.[6]
The spacecraft is equipped with seven scientific instruments,[7][8] including the Tracking Ultraviolet Set Up system (TUS) designed to measure fluorescence light radiated by EAS (Extensive Air Showers) of Ultra High Energy Cosmic Rays (UHECR) in the Earth atmosphere as well as for transients' studies within UV-range. This was the first space based instrument dedicated to these phenomena. The TUS-project started in 2001.[9]
Ultraviolets
[edit | edit source]


Ultraviolet lamps are also used in analyzing minerals and gems. Materials may look the same under visible light, but fluoresce to different degrees under ultraviolet light, or may fluoresce differently under short wave ultraviolet versus long wave ultraviolet.
Ultraviolet lamps may cause certain minerals to fluoresce, and is a key tool in prospecting for tungsten mineralisation.
"Between 190 and 1700 nm, the ordinary refractive index varies roughly between 1.9 and 1.5, while the extraordinary refractive index varies between 1.6 and 1.4.[10]
Under longwave (365 nm) ultraviolet light, diamond may fluoresce a blue, yellow, green, mauve, or red of varying intensity. The most common fluorescence is blue, and such stones may also phosphoresce yellow—this is thought to be a unique combination among gemstones. There is usually little if any response to shortwave ultraviolet.
Visuals
[edit | edit source]
Def. the "emission of light (or other electromagnetic radiation) by a material when stimulated by the absorption of radiation or of a subatomic particle"[11] is called fluorescence.
Fluorescence is the emission of light by a substance that has absorbed light or other electromagnetic radiation. It is a form of luminescence. In most cases, the emitted light has a longer wavelength, and therefore lower energy, than the absorbed radiation. However, when the absorbed electromagnetic radiation is intense, it is possible for one electron to absorb two photons; this two-photon absorption can lead to emission of radiation having a shorter wavelength than the absorbed radiation. The emitted radiation may also be of the same wavelength as the absorbed radiation, termed "resonance fluorescence".[12]
The most striking examples of fluorescence occur when the absorbed radiation is in the ultraviolet region of the spectrum, and thus invisible to the human eye, and the emitted light is in the visible region.
The common fluorescent lamp relies on fluorescence. Inside the glass tube is a partial vacuum and a small amount of mercury. An electric discharge in the tube causes the mercury atoms to emit ultraviolet light. The tube is lined with a coating of a fluorescent material, called the phosphor, which absorbs the ultraviolet and re-emits visible light. Fluorescent lighting is more energy-efficient than incandescent lighting elements. However, the uneven spectrum of traditional fluorescent lamps may cause certain colors to appear different than when illuminated by incandescent light or daylight. The mercury vapor emission spectrum is dominated by a short-wave UV line at 254 nm (which provides most of the energy to the phosphors), accompanied by visible light emission at 436 nm (blue), 546 nm (green) and 579 nm (yellow-orange). These three lines can be observed superimposed on the white continuum using a hand spectroscope, for light emitted by the usual white fluorescent tubes. These same visible lines, accompanied by the emission lines of trivalent europium and trivalent terbium, and further accompanied by the emission continuum of divalent europium in the blue region, comprise the more discontinuous light emission of the modern trichromatic phosphor systems used in many compact fluorescent lamp and traditional lamps where better color rendition is a goal.[13]
Moon
[edit | edit source]
The Chandra X-ray Observatory, right image, detects X-rays from the Moon. These X-rays are produced by fluorescence when solar X-rays bombard the Moon's surface. Close inspection of the Chandra X-ray image shows a region of X-rays in the dark region trending toward the lower left corner of the X-ray image. These X-rays only appear to come from the Moon. Instead, they originate from radiation of the Earth's geocorona (an extended outer atmosphere) through which orbiting spacecraft such as the Chandra satellite move.
Mars
[edit | edit source]

"Far-ultraviolet spectra ... Mars in the range 820-1840 Å at ~4 Å resolution were obtained on 1995 March 13 and 12, respectively, by the Hopkins Ultraviolet Telescope (HUT), which was part of the Astro-2 observatory on the space shuttle Endeavour. Longward of 1250 Å, the spectra ... are dominated by emission of the CO fourth positive (A1Π-X1Σ+) band system and strong O I and C I multiplets. ... The Ar I λλ1048, 1066 doublet is detected only in the spectrum of Mars ... CO fluorescence in both the B−X (0,0) and C−X (0,0) Birge-Hopfield bands is identified in ... Mars ... Below 2000 Å, the ultraviolet dayglow of ... Mars is dominated by emissions of carbon monoxide and carbon (Durrance 1981; Fox 1992)."[14]
On the right is an ultraviolet photograph of Mars. It shows clouds and other aspects of the atmosphere.
The image at left is an X-ray image of Mars. X-radiation from the Sun excites oxygen atoms in the Martian upper atmosphere, about 120 km above its surface, to emit X-ray fluorescence. A faint X-ray halo that extends out to 7,000 km above the surface of Mars has also been found.[15] The Chandra X-ray Observatory image on the right is the first look at X-rays from Mars. If X-ray astronomy was the first astronomy to view Mars, the conclusion that the X-rays are fluorescence rather than emission is important. The first class of X-ray sources may be fluorescence sources rather than emission sources.
Jupiter
[edit | edit source]
The "image of Jupiter [at right] shows concentrations of auroral X-rays near the north and south magnetic poles."[16] The Chandra X-ray Observatory accumulated X-ray counts from Jupiter for its entire 10-hour rotation on December 18, 2000. Note that X-rays from the entire globe of Jupiter are detected.
The X-rays from the poles of Jupiter are not fluorescent X-rays but emission X-rays. Those from the main portion of Jupiter may be fluorescence with some reflectance.
Saturn
[edit | edit source]
The X-ray astronomy image of Saturn is on the left in the composite at right. The Chandra X-ray Observatory "image of Saturn held some surprises for the observers. First, Saturn's 90 megawatts of X-radiation is concentrated near the equator. This is different from a similar gaseous giant planet, Jupiter, where the most intense X-rays are associated with the strong magnetic field near its poles. Saturn's X-ray spectrum, or the distribution of its X-rays according to energy, was found to be similar to that of X-rays from the Sun. This indicates that Saturn's X-radiation is due to the reflection of solar X-rays by Saturn's atmosphere. The intensity of these reflected X-rays was unexpectedly strong. ... The optical image of Saturn is also due to the reflection of light from the Sun - visible wavelength light in this case - but the optical and X-ray images obviously have dramatic differences. The optical image is much brighter, and shows the beautiful ring structures, which were not detected in X-rays. This is because the Sun emits about a million times more power in visible light than in X-rays, and X-rays reflect much less efficiently from Saturn's atmosphere and rings."[17]
"[T]he soft X-ray emissions of Jupiter (and Saturn) can largely be explained by scattering and fluorescence of solar X-rays."[18]
The second image at the right, "taken by the Chandra x-ray telescope, reveals that the rings of Saturn sparkle; in this x-ray/optical composite, they are visible as blue dots. This radiation’s source is likely fluorescence caused by solar x-rays as they strike oxygen atoms in the water molecules of the planet’s icy rings. As the image shows, most of the ring’s x-rays originate in the B ring—the bright white inner ring visible in this optical image—which is approximately 25,000 kilometers wide and 40,000 kilometers above the planet’s surface. X-rays may also be concentrated on Saturn’s left side, possibly because of their association with shadows in the planet’s rings that are known as spokes, or possibly as a result of the additional solar fluorescence caused by the transient ice clouds that produce spokes. Other Chandra observations of Saturn show that the x-ray brightness of the rings varies significantly from one week to the next."[19]
Comets
[edit | edit source]The Sun emits visual radiation that may reflect off a comet's tail. The coronal cloud in close proximity to the Sun also emits X-rays that produce visual fluorescence from gases in a comet's coma and tail.
Galaxies
[edit | edit source]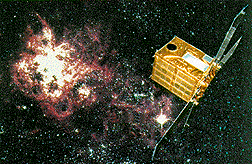
Some "60 spectra of 27 Seyfert galaxies [were observed] with the Ginga Large Area proportional Counter (LAC). The 2-10 keV continuum is found to be compatible with previous spectral surveys, but a spectral flattening, or 'hard tail', is evident above 10 keV. Excess absorption over the Galactic column density is found in around half of the soures, with an equivalent hydrogen column density NH = 1021-22 cm-2. Spectral features are found to be common, with all but two of the sources showing evidence for an iron K𝛂 emission line. The mean energy of the line, at around 6.4 keV, indicates an origin via fluorescence in near-neutral material."[20]
Calciums
[edit | edit source]

Calcium (Ca) has green emission lines at 526.170 nm and 526.556 nm as observed in solar limb faculae.[21]
As of 1977, "model calculations cannot reproduce the observed breadth of the Ca II λ3933 line in Da,F stars like Ross 627 without appealing to an unknown line-broadening mechanism".[22]
Calcium has a line occurring in the solar corona at 408.63 nm of Ca XIII.[23]
Calcium (Ca I) has two absorption bands, 422.673±4.5 nm and 430.253±0.6.[24] The second has an excitation potential of 1.89 eV.[24]
Calcium (Ca II) has an absorption band, 393.366±55.0.[24]
"Heinrich Layers are found in the North Atlantic Ocean as well-constrained markers of catastrophic iceberg surges from the Pan-Atlantic ice sheets during the last glacial cycle. Their physical and geochemical characteristics [...] are predominantly due to the source sediments of the ice-rafted debris (IRD) on the one hand (magnetic susceptibility, color, carbonate content) and the response of the palaeo-environment on the other hand (carbonate content, foraminiferal assemblage)."[25]
"Sediment cores in the Porcupine Seabight (West off Ireland) have shown the presence of Heinrich Events without the diagnostic changes in magnetic susceptibility (MS) [...] the concentration of ice-rafted debris (commonly referred to as the fraction > 150 μm) increases towards the culmination of HL2, marked by an increase in MS, [X-ray fluorescence] XRF Ca and the percentage of N. pachyderma s."[25]
The "zone where the density increases is marked by a cloud of fine and highly dense particles surrounding the IRD. [The] fine clayey “background” matrix throughout the core [consists] of zoned dolomites. [...] the mineralogical analyses [suggest] a predominant volcanic source for the magnetic susceptibility. [Both] XRF Fe and Ti show significant decreases near the HL culmination".[25]
Diamonds
[edit | edit source]Under longwave (365 nm) ultraviolet light, diamond may fluoresce a blue, yellow, green, mauve, or red of varying intensity. The most common fluorescence is blue, and such stones may also phosphoresce yellow—this is thought to be a unique combination among gemstones. There is usually little if any response to shortwave ultraviolet.
International Space Station
[edit | edit source]The Extreme Universe Space Observatory (EUSO) [on the third right] is the first Space mission concept devoted to the investigation of cosmic rays and neutrinos of extreme energy (E > 5×1019
eV). Using the Earth's atmosphere as a giant detector, the detection is performed by looking at the streak of fluorescence produced when such a particle interacts with the Earth's atmosphere.
MetOp-A & -B spacecraft
[edit | edit source]
The first atmospheric contributions by Metop-A were made by the Global Ozone Monitoring Experiment-2 (GOME-2), a scanning spectrometer on board the satellite. GOME-2, designed by German Aerospace Center (DLR) and developed by SELEX Galileo as the successor of European Remote-Sensing Satellite (ERS-2)'s GOME (1995), provided coverage of most areas of planet Earth measuring the atmospheric ozone, the distribution of surface ultraviolet radiation, and the amount of nitrogen dioxide (NO2).[26] In addition, sun-induced chlorophyll fluorescence, a proxy for gross primary production, can be observed using the GOME-2 instrument.[27][28] The GOME-2 instrument provides a second source of ozone observations that supplement data from the SBUV/2 ozone instruments on the NOAA-18 and NOAA-19 satellites, which are part of the IJPS.[29]
Technology
[edit | edit source]Condensed noble gases, most notably liquid xenon and liquid argon, are excellent radiation detection media. They can produce two signatures for each particle interaction: a fast flash of light (scintillation) and the local release of charge (ionisation). In two-phase xenon – so called since it involves liquid and gas phases in equilibrium – the scintillation light produced by an interaction in the liquid is detected directly with photomultiplier tubes; the ionisation electrons released at the interaction site are drifted up to the liquid surface under an external electric field, and subsequently emitted into a thin layer of xenon vapour. Once in the gas, they generate a second, larger pulse of light (electroluminescence or proportional scintillation), which is detected by the same array of photomultipliers. These systems are also known as xenon 'emission detectors'.[30]
The basic set-up consists of 1600 water tanks (water Cherenkov Detectors, similar to the Haverah Park experiment) distributed over 3,000 square kilometres (1,200 sq mi), along with four atmospheric fluorescence detectors (similar to the High Resolution Fly's Eye) overseeing the surface array.
The Pierre Auger Observatory is unique in that it is the first experiment that combines both ground and fluorescence detectors at the same site thus allowing cross-calibration and reduction of systematic effects that may be peculiar to each technique. The Cherenkov detectors use three large photomultiplier tubes to detect the Cherenkov radiation produced by high-energy particles passing through water in the tank. The time of arrival of high-energy particles from the same shower at several tanks is used to calculate the direction of travel of the original particle. The fluorescence detectors are used to track the particle shower's glow on cloudless moonless nights, as it descends through the atmosphere.
The bubble chamber reveals the tracks of subatomic particles as trails of bubbles in a superheated liquid, usually liquid hydrogen. Bubble chambers can be made physically larger than cloud chambers, and since they are filled with much-denser liquid material, they reveal the tracks of much more energetic particles.
The field of neutrino astronomy is still very much in its infancy – the only confirmed extraterrestrial sources so far are the Sun and supernova SN1987A. Various detection methods have been used. Super Kamiokande is a large volume of water surrounded by phototubes that watch for the Cherenkov radiation emitted when an incoming neutrino creates an electron or muon in the water. The Sudbury Neutrino Observatory is similar, but uses heavy water as the detecting medium. Other detectors have consisted of large volumes of chlorine or gallium which are periodically checked for excesses of argon or germanium, respectively, which are created by neutrinos interacting with the original substance. [...] Borexino uses a liquid pseudocumene scintillator also watched by phototubes while the proposed NOνA detector will use liquid scintillator watched by avalanche photodiodes.
Scintillation neutron detectors include liquid organic scintillators,[31] crystals,[32][33] plastics, glass[34] and scintillation fibers.[35]
See also
[edit | edit source]References
[edit | edit source]- ↑ Stokes, G. G. (1852). "On the Change of Refrangibility of Light". Philosophical Transactions of the Royal Society of London 142: 463–562. doi:10.1098/rstl.1852.0022.
- ↑ K. Przibram (1935). "Fluorescence of Fluorite and the Bivalent Europium Ion". Nature 135 (3403): 100. doi:10.1038/135100a0.
- ↑ "idrialite". San Francisco, California: Wikimedia Foundation, Inc. 20 June 2013. Retrieved 2015-01-09.
- ↑ P Morrison (1967). "Extrasolar X-ray Sources". Annual Review of Astronomy and Astrophysics 5 (1): 325–50. doi:10.1146/annurev.aa.05.090167.001545.
- ↑ Kupperian JE Jr, Friedman H (1958). "Experiment research US progr. for IGY to 1.7.58". IGY Rocket Report Ser. (1): 201.
- ↑ "Soyuz prepared for first flight from Siberian cosmodrome". Spaceflight Now. Retrieved 21 March 2016.
- ↑ "MVL-300 (Mikhailo Lomonosov)". Gunter's Space Page. Retrieved 21 March 2016.
- ↑ "Космический аппарат "Ломоносов"" [The spacecraft "Lomonosov"] (in Russian). VNIIEM. Retrieved 21 March 2016.
- ↑ Khrenov, B.A.; Garipov, G.K.; Kaznacheeva, M.A.; Klimov, P.A.; Panasyuk, M.I.; Petrov, V.L.; Sharakin, S.A.; Shirokov, A.V. et al. (2020). "An extensive-air-shower-like event registered with the TUS orbital detector". Journal of Cosmology and Astroparticle Physics 2020 (3): 033. doi:10.1088/1475-7516/2020/03/033.
- ↑ D.W. Thompson (1998). "Determination of optical anisotropy in calcite from ultraviolet to mid-infrared by generalized ellipsometry". Thin Solid Films 313–4 (1-2): 341–6. doi:10.1016/S0040-6090(97)00843-2.
- ↑ SemperBlotto (10 September 2006). fluorescence. San Francisco, California: Wikimedia Foundation, Inc. https://en.wiktionary.org/wiki/fluorescence. Retrieved 2017-04-10.
- ↑ Principles Of Instrumental Analysis F.James Holler, Douglas A. Skoog & Stanley R. Crouch 2006
- ↑ Tom Harris. How Fluorescent Lamps Work. Discovery Communications. http://home.howstuffworks.com/fluorescent-lamp.htm. Retrieved 27 June 2010.
- ↑ Paul D. Feldman; Eric B. Burgh; Samuel T. Durrance; Arthur F. Davidsen (July 2000). "Far-Ultraviolet Spectroscopy of Venus and Mars at 4 Å Resolution with the Hopkins Ultraviolet Telescope on Astro-2". The Astrophysical Journal 538 (1): 395-400. doi:10.1086/309125.
- ↑ K. Dennerl (November 2002). "Discovery of X-rays from Mars with Chandra". Astronomy & Astrophysics 394 (11): 1119-28. doi:10.1051/0004-6361:20021116.
- ↑ NASA/CXC/SWRI/G.R.Gladstone (27 February 2002). Jupiter Hot Spot Makes Trouble For Theory. Cambridge, Massachusetts: Harvard-Smithsonian Center for Astrophysics. http://chandra.harvard.edu/photo/2002/0001/. Retrieved 2012-07-11.
- ↑ Samantha Harvey (August 19, 2008). X-Ray Saturn. NASA. http://solarsystem.nasa.gov/multimedia/display.cfm?Category=Planets&IM_ID=1443. Retrieved 2012-07-21.
- ↑ G. Branduardi-Raymont, A. Bhardwaj, R.F. Elsner, G.R. Gladstone, G. Ramsay, P. Rodriguez, R. Soria, J.H. Waite Jr., T.E. Cravens (June 2007). "Latest results on Jovian disk X-rays from XMM-Newton". Planetary and Space Science 55 (9): 1126-34. doi:10.1016/j.pss.2006.11.017. http://arxiv.org/pdf/astro-ph/0609758. Retrieved 2013-05-23.
- ↑ Chandra X-ray Observatory Center (2003). click! Photography Changes Everything. Cambridge, Massachusetts USA: Smithsonian Astrophysical Observatory. http://click.si.edu/Image.aspx?image=433&story=31&back=ImageIndex&page=1. Retrieved 2014-05-31.
- ↑ K. Nandra & K. A. Pounds (15 May 1994). "GINGA Observations of the X-Ray Spectra of Seyfert Galaxies". Monthly Notices of the Royal Astronomical Society 268 (2): 405-29. http://adsabs.harvard.edu/full/1994MNRAS.268..405N7. Retrieved 4 June 2021.
- ↑ G. Stellmacher; E. Wiehr (August 1991). "Geometric line elevation in solar limb faculae". Astronomy and Astrophysics 248 (1): 227-31.
- ↑ H. L. Shipman (April 1977). "Masses, radii, and model atmospheres for cool white-dwarf stars". The Astrophysical Journal 213 (4): 138-44. doi:10.1086/155138.
- ↑ P. Swings (July 1943). "Edlén's Identification of the Coronal Lines with Forbidden Lines of Fe X, XI, XIII, XIV, XV; Ni XII, XIII, XV, XVI; Ca XII, XIII, XV; a X, XIV". The Astrophysical Journal 98 (07): 116-28. doi:10.1086/144550.
- ↑ 24.0 24.1 24.2 Kozo Sadakane; Minoru Ueta (August 1989). "Abundance Analysis of Sirius in the Blue-Violet Region". Publications of the Astronomical Society of Japan 41 (2): 279-88.
- ↑ 25.0 25.1 25.2 D. Van Rooij; N. Zaazi; N. Fagel; M. Boone; V. Cnudde; J. Dewanckele; H. Pirlet; U. Rohl et al. (2009). "3D anatomy of Heinrich Layer 2". Geophysical Research Abstracts 11 (EGU2009-4809-1): 1. http://meetingorganizer.copernicus.org/EGU2009/EGU2009-4809-1.pdf. Retrieved 2014-09-29.
- ↑ Spaceflight, a publication of the British Interplanetary Society, Volume 49, Number 5, May 2007, page 166.
- ↑ Joiner, J.; Guanter, L.; Lindstrot, R.; Voigt, M.; Vasilkov, A. P.; Middleton, E. M.; Huemmrich, K. F.; Yoshida, Y. et al. (25 October 2013). "Global monitoring of terrestrial chlorophyll fluorescence from moderate-spectral-resolution near-infrared satellite measurements: methodology, simulations, and application to GOME-2". Atmospheric Measurement Techniques 6 (10): 2803–2823. doi:10.5194/amt-6-2803-2013.
- ↑ Koren, Gerbrand; van Schaik, Erik; Araújo, Alessandro C.; Boersma, K. Folkert; Gärtner, Antje; Killaars, Lars; Kooreman, Maurits L.; Kruijt, Bart et al. (19 November 2018). "Widespread reduction in sun-induced fluorescence from the Amazon during the 2015/2016 El Niño". Philosophical Transactions of the Royal Society B: Biological Sciences 373 (1760): 20170408. doi:10.1098/rstb.2017.0408.
- ↑ "NOAA-N Prime" (PDF). NP-2008-10-056-GSFC. NASA Goddard Space Flight Center. 16 December 2008. Archived from the original (PDF) on 16 February 2013. Retrieved 8 October 2010.
- ↑ B. A. Dolgoshein, V. N. Lebedenko & B. I. Rodionov, "New method of registration of ionizing-particle tracks in condensed matter", JETP Lett. 11(11): 351 (1970)
- ↑ Yousuke, I.; Daiki, S.; Hirohiko, K.; Nobuhiro, S.; Kenji, I. (2000). "Deterioration of pulse-shape discrimination in liquid organic scintillator at high energies". Nuclear Science Symposium Conference Record, Volume: 1 (IEEE) 1: 6/219–6/221 vol.1. doi:10.1109/NSSMIC.2000.949173. ISBN 0-7803-6503-8.
- ↑ Kawaguchi, N.; Yanagida, T.; Yokota, Y.; Watanabe, K.; Kamada, K.; Fukuda, K.; Suyama, T.; Yoshikawa, A. (2009). "Study of crystal growth and scintillation properties as a neutron detector of 2-inch diameter eu doped LiCaAlF6 single crystal". Nuclear Science Symposium Conference Record (NSS/MIC) (IEEE): 1493–1495. doi:10.1109/NSSMIC.2009.5402299. ISBN 978-1-4244-3961-4.
- ↑ Example crystal scintillator based neutron monitor.
- ↑ Bollinger, L.M.; Thomas, G.E.; Ginther, R.J. (1962). "Neutron Detection With Glass Scintillators". Nuclear Instruments and Methods 17: 97–116.
- ↑ Miyanaga, N.; Ohba, N.; Fujimoto, K. (1997). "Fiber scintillator/streak camera detector for burn history measurement in inertial confinement fusion experiment". Review of Scientific Instruments 68 (1): 621–623. doi:10.1063/1.1147667.